COMPARING THE IN SITU AND EX SITU MICROBIOME OF THREE BERMUDA CORAL SPECIES FOR MICROBIAL COMPOSITION AND ABUNDANCE
Abstract
Coral health studies are often preformed within simulated ocean environments which typically only contain the coral, seawater, and light. However, flourishing reef environments encompass a diverse system, including many other factors that dictate coral health (Bourne et al. 2016; Wegley et al. 2007; Rohwer et al. 2002). There has been very little research conducted comparing the microbiome of mesocosm and in situ coral colonies. This study is focused on extracting, observing, and quantifying overall microbial abundance and composition in the microbiome of the coral. This study will examine three species of coral (Porites astreoides, Montastraea cavernosa, Diploria labyrinthiformis) to compare microbial communities between environments. In situ colonies were harvested using SCUBA at Bailey’s Bay Flats, Bermuda, and mesocosm coral specimens were from the Bermuda Institute of Ocean Sciences (BIOS) mesocosm after being within that environment for approximately four weeks. A significant difference was exhibited in the microbial abundance between in situ and mesocosm coral species (p= 4.865e-05, Kruskal Wallis). Porites astreoides (p=0.0185, Kruskal Wallis), Montastraea cavernosa (p=0.0023, Kruskal Wallis), and Diploria labyrinthiformis (p=0.0163, Kruskal Wallis) all exhibited a significant difference between environments. These results suggest that in situ microbial abundance is statistically higher than corals within aquaria environments. The most common microbe that was probed for among the in situ and mesocosm P. astreoides was Vibrio, M. cavernosa was Endozoicomona, D. labyrinthiformis was Flavobacteria (in situ) and Vibrio (Mesocosm). There was no significant difference in bacterial composition across environments and species (R2= 0.04689, PERMANOVA). These results show that even though there is a statistical difference in microbial abundance between the two environments, the microbes within remain similarly proportioned. The data showed no significant difference in zooxanthellae between environmental variables (p= 0.8055, Kruskal Wallis). This study provides a small snapshot into the complex bacterial abundance and composition held within the coral microbiome.
Introduction
The coral holobiont is comprised of a unique accumulation of microbial eukaryotes, fungi and protozoa, Bacteria, Archaea and viruses (Wegley et al. 2007; Rohwer et al. 2002). These microbes are important for the coral’s health and biological processes (Galand et al. 2018; Wegley et al. 2007; Rohwer et al. 2002). Corals are made up of many microhabitats for microbes including the surface mucopolysaccharide layer (SML), epidermis, gastrodermis, gastric cavity, and coral skeleton (Bourne et al. 2016). The corals SML is secreted by cells in the epidermis and is comprised of glycoproteins containing enzyme-rich polypeptides and sulphated oligosaccharides which are secreted by the endosymbiotic Symbiodinium spp. that resides within the coral endodermis, also known as zooxanthellae (Bourne et al. 2016; Wegley et al. 2007; Rohwer et al. 2002). This mucus layer supports a microbial community containing 106–108 cells per milliliter (Garren & Azam 2012), creates a protective layer of antibacterial compounds which shield the coral tissue from pathogens (Ritchie 2006; Broderick et al. 2008), supplies the coral with essential nutrients (Wild et al 2004), and produces ultraviolet light–absorbing pigments for the purpose of protecting against solar radiation (Ravindran et al. 2013). It is also suspected that coral microbiome change depending on what environmental conditions they are subjected to in order to provide resistance to specific pathogens (Wegley et al. 2007; Rohwer et al. 2002).
The microbes within the coral holobiont perform important nutrient cycling within the coral animal. These microbes cycle carbon, nitrogen, and sulphur along with other important nutrients (Bourne et al. 2016). Stony corals (Scleractinia) can acquire up to 60% of their carbon requirements through heterotrophy and/or up to 100% of their carbon requirements through photoautotrophy (Bourne et al. 2016; Palardy 2008; Falkowski et al. 1993). In recent studies, it has been shown that microorganisms transform and pass nutrients within the different components of the coral holobiont (Bourne et al. 2016; Kimes et al. 2010). Nitrogen is limited within coral reef ecosystems, forcing the evolution of strategies to derive nitrogen from heterotrophic feeding and inorganic sources (Bourne et al. 2016; Falkowski et al. 1993). Zooxanthellae possess RUBISCO and nitrate reductase enzymes which allow them to convert in-organic forms such as substrate into organic forms of nitrogen (Bourne et al. 2016; Yellowlees et al. 2008). Microbes within the coral holobiont then regulate the amount of nitrogen within the coral animal through nitrogen fixation, nitrification, and denitrification (Bourne et al. 2016: Lema et al. 2012; Siboni et al. 2008; Wegley et al. 2007). Bacteria and archaea that are capable of fixing nitrogen are known as diazotrophs (Bourne et al. 2016). Diazotrophs can also supplement the coral holobiont with nitrogen in the form of ammonia (Bourne et al. 2016). A huge contributor to sulphur within the coral holobiont is incorporated into cysteine and methionine and converted to dimethyl-sulfoniopropionate (DMSP) (Stefels 200; Van Alstyne 2006). Coral-derived DMSP is readily available carbon source for coral microorganisms and likely is a main player in forming associations within the coral holobiont (Bourne et al. 2016). Acquisition of these nutrients can be greatly altered through environmental stress events (Bourne et al. 2016).
The coral microbiome and the microorganisms that populate it are critical to the hosts fitness, survival, and health (Galand et al. 2018; Wegley et al. 2007; Rohwer et al. 2002). Coral health studies occur across various countries and are often within artificial environments, like laboratory tanks, mesocosm rigs, and wet labs. These simulated environments are often only comprised of the coral species of interest, seawater, and light. However, flourishing reef environments encompass a diverse and complex system, including many other factors that help dictate coral health (Bourne et al. 2016; Wegley et al. 2007; Rohwer et al. 2002). The few studies that have been conducted comparing captive coral microbiomes and in situ suggest found that microbiomes differ between in-situ and captive (Galand et al. 2018; Röthig et al. 2017; Pratte et al., 2015; Schöttner et al. 2009; Kooperman et al. 2007). One way to learn more about how corals cope in these captive habitats is to continue studying their microbiome.
This study is focused on extracting, observing, and quantifying overall microbial abundance and composition in the microbiome of the coral animal and will be using three species to compare microbial communities of the same species in situ and mesocosm environments. It is predicted that coral microbial abundance in the microbiome will be statistically different across mesocosm and in situ specimens. This is based on prior research that suggests that microbial abundance in the coral microbiome between captive and in situ corals is always different (Galand et al. 2018; Röthig et al. 2017; Pratte et al., 2015; Schöttner et al. 2009; Kooperman et al. 2007). The microbiota will most likely be different in the mesocosm corals compared to the in situ corals because the microbiota of the coral microbiome is known to when moved to mesocosm environments (Pratte et al. 2015). This indicates that the mesocosm specimens will have differing microbiota to their in situ replicates. The zooxanthellae of mesocosm is predicted to be higher when compared to the in situ specimens because of differing light qualities and photoadaptation was expected to be observed among the corals within the mesocosm setting because of the different light quality. It is thought that each coral species will have comparable microbial abundances while differing across mesocosm and in situ environments. This hypothesis is based on prior research indicating that there might be a core coral microbiome (Chu et al. 2016). If there is a relationship between microbial abundance and a given coral environment, this could have implications on how lab coral specimens should be maintained. This study hopes to assist in the interpretation of coral physiology in response to mesocosm conditions.
Methods
Species Description
Porites astreoides, Montastraea cavernosa, and Diploria labyrinthiformis (phyla Cnidaria, class Anthozoa, order Scleractinia) are all hermatypic scleractinian coral that are present within virtually all of Bermuda’s reef systems. P. astreiodes and D. labyrinthiformis have an assortment of differing morphologies it can acquire (encrusting, mound, and plate) providing this species with morphological plasticity when it comes to growth in differing environmental structures (Chornesky 1987). M. cavernosa can acquire those same morphologies, excluding plating. All three of these corals occur in an extensive number of depths and habitats inhabiting a wide variety of the world’s Caribbean reefs (Chornesky 1987). Like other reef building corals, P. astreoides, M. cavernosa, and D. labyrinthiformis partakes in a mutualistic relationship with an endosymbiotic dinoflagellate known as zooxanthellae.


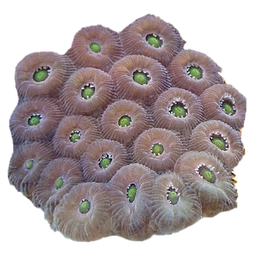
Diploria labyrinthiformis
Montastraea cavernosa
Porites astreoides
Colony Harvesting and Coral Microbiome Sampling
The mesocosm colonies were within the BIOS aquaria environment for approximately four weeks before sampling occurred. To obtain the in situ colonies with the use of a permit, corals of these three species were harvested at site Baileys Bay Flats, Bermuda using SCUBA. Small portions of four separate colonies per three species were extracted from the larger colony with the use of a hammer and chisel. Each coral fragment was then placed in a labeled bag that corresponds with the colony it was taken from. These fragments were then placed into a cooler with seawater from the study site with the plastic bags open for water flow. The coral microbiome was then immediacy sampled once returning to the station.
The coral microbiome will be extracted from mesocosm colonies (n = 4) and in-situ colonies (n= 4) using a water pick with sterile seawater (40mL). After, the corals were returned to the holding aquaria with flow through seawater. This 40mL sample will then be vortexed for five minutes. After vortexting, two mL of sample was be diluted with 8 mL of filtered seawater and sonicated for 15 minutes. Then this diluted sample was be fixed with 1 mL filtered formalin to create a 10% concentration (eleven mL of sample). If the specimen was part of the in situ group, this sample was then diluted once more using two mL of the sonicated sample and 8 mL of filtered seawater, then vortexed once again to conform mixture of the sample. 5 mL of this solution was then filtered through a 2.0um PC Membrane filter using a vacuum (5-7 mm Hg) to separate out any larger microorganisms. This filter was then saved and used for zooxanthellae counts. Initial tests were conducted to determine the best method for extracting the microbiome and the best volume needed to ensure statistically significant microbial abundance measurements.
Microbial and Zooxanthellae Abundance
3 mL of the 11 mL sample was filtered through a polycarbonate 0.2µm 25mm filter previously stained with Irgalan black (SIGMA-Aldrich, St. Louis, MO USA) under light vacuum (5-7 mm Hg). The filters were then stained in darkness with 4’, 6-di-amidino-2-phenylindole dihydrochloride (5 ug ml-1 DAPI, SIGMA-Aldrich, St. Louis, MO USA) and placed on a microscope slide using Resolve® immersion oil. These samples were then stored at -80C until further use (Porter and Feig, 1980). Total microbial and zooxanthellae abundance was determined using an AX70 epifluorescent microscope (Olympus, Tokyo, Japan) under ultraviolet excitation at 100x magnification. At least 400 cells (10 fields) were counted for cell abundance and normalized per mL of seawater and per surface area of each coral fragment.
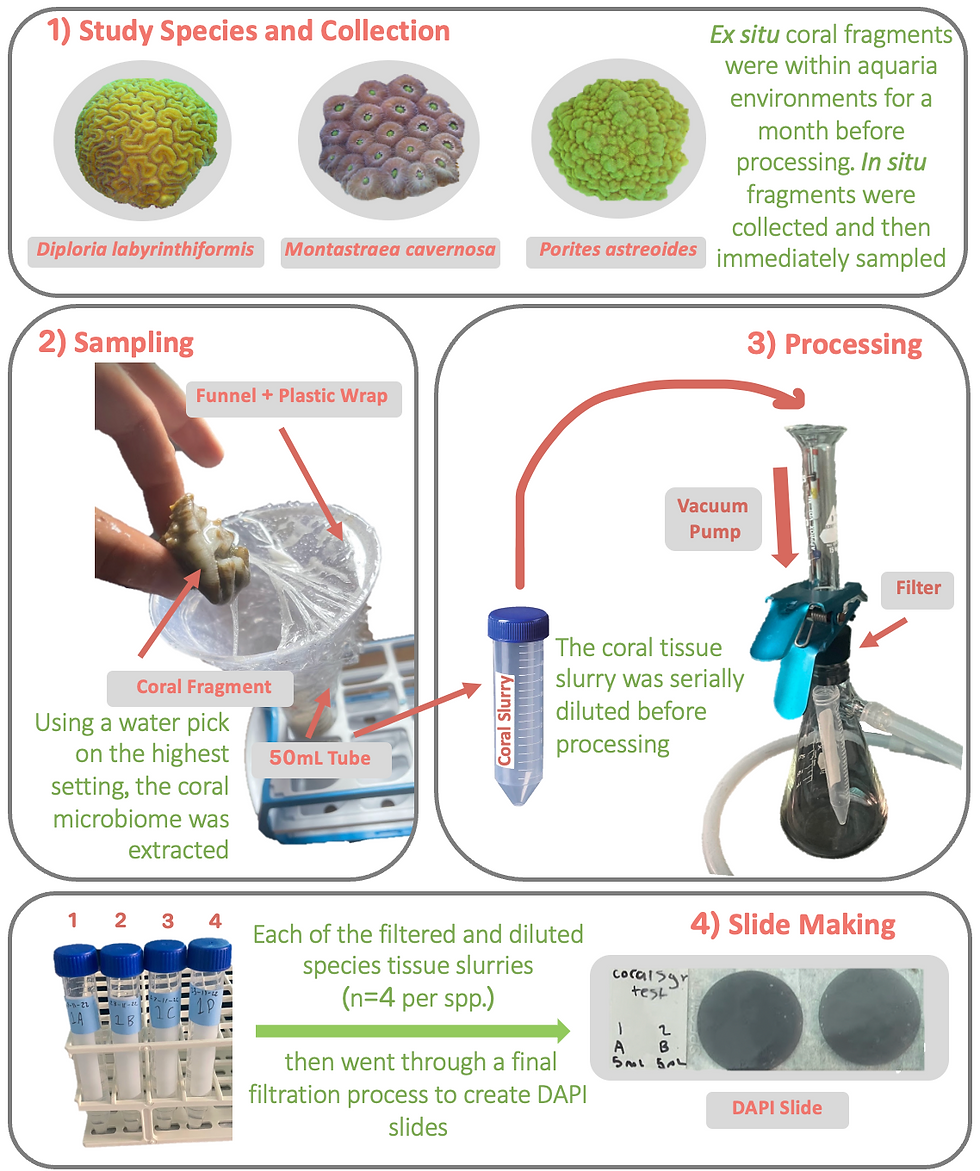
Determining Microbial Composition
Fluorescence in situ hybridization (FISH) was performed on all three species across both environments to compare the microbes within the coral microbiome (Moter & Göbel et al. 2000). Each sample was fixed with 10% formalin and filtered through a 2.0um PC Membrane filter using a vacuum (5-7 mm Hg) to separate out any larger microorganisms. These filters are then disposed of. Then, each sample is filtered through a 0.2µm 25mm filter and then mounted to a slide with stickers. Each filter was then cut up into six pieces and mounted on to separate slides with the stickers. Each of these slides represents a different bacteria species that is being tested for (Pseudoalteromonas, Endozoicomonas, Flavobacteria, Rhodobacteracea, Alteromonas, Vibrio). These filters were then washed in 95% ethanol. Each sample was probed with 40µl of 2ng/µl probe in hybridization solution and a cover slip was attached. After, these samples were placed into a hybridisation oven for 16 hours. These ovens were set to 46°C (Pseudoalteromonas, Endozoicomonas, Flavobacteria) and 37°C (Rhodobacteracea, Alteromonas, Vibrio). After the 16 hour period, a coplin jar with hybridization wash solution was placed into a water bath and brought up to temperature. Each sample then sat in two separate Jar washes for 10 minutes each (20 min total), then be dried and mounted onto the slide with 20µl DAPI/citiflour (1:2) mixture using a cover slide.
Samples were placed on a microscope slide using Resolve® immersion oil after FISH protocol was complete. Total microbial abundance of each bacteria species (Pseudoalteromonas, Endozoicomonas, Flavobacteria, Rhodobacteracea, Alteromonas, Vibrio) was determined using an AX70 epifluorescent microscope (Olympus, Tokyo, Japan) under ultraviolet excitation at 100x magnification. These samples were then stored at -80C until further use (Porter and Feig, 1980). At least 200 cells (5 fields) were counted for cell abundance and normalized per mL of seawater and per surface area of each coral fragment.
Data Analysis
Bacterial and zooxanthellae abundance were calculated using the following formula:
((C)(F)(D))/((V)(FC)) = Cells/ml
In the formula above, C is average cells/field, F is the F value, D is the dilution correction, V is volume filtered and FC is field counted. All calculations will be carried out in Microsoft Excel. Microbial abundances between in situ and mesocosm specimens across three different species was assessed using Kruskal Wallis statistical analysis. This test was chosen because if was found that the data set was non-parametric, which was found using a Shapiro-Wilk test. To test for where significance between variables came from, a Post-Hoc Dunn test was conducted. For differences in bacterial composition between environment and species, a PERMANOVA was performed. Zooxanthellae abundances between in situ and mesocosm specimens across three different species was assessed using Kruskal Wallis statistical analysis. This test was chosen because if was found that the data set was non-parametric, found using a Shapiro-Wilk test.
Results
There was a significant difference in microbial abundance between in situ and mesocosm coral colonies (p= 4.865e-05, Kruskal Wallis, Figure 1). This reveled that the coral colonies in situ had a statistically higher microbial abundance compared to the mesocosm specimens. This was observed across all species (Porites astreoides, p=0.0185; Montastraea cavernosa, p=0.0023; Diploria labyrinthiformis, p=0.0163; Post-Hoc Dunn, Figure 1). Microbial abundance in situ showed no significant difference across species (p=>0.05, Post-Hoc Dunn, Figure 1). Microbial abundance in the mesocosm also showed no significant difference across species (p=>0.05, Post-Hoc Dunn, Figure 1). The microbial composition of the mesocosm Porites astreoides was 18.13% Vibrio, 14.74% Pseudoalteromonas, 11.32% Alteromonas, 10.83% Endozoicomonas, 9.73% Rhodobacteracea, 6.58% Flavobacteria, with 28.67% being unprobed (Figure 3). The bacterial community among the in situ Porites astreoides was 31.1% Vibrio, 24.61% Endozoicomonas, 8.05% Rhodobacteracea, 6.91% Pseudoalteromonas, 5.77% Flavobacteria, 4.5% Alteromonas, with 19.06% being unprobed (Figure 3). Mesocosm Montastraea cavernosa was 27.59% Endozoicomonas, 3.96% Vibrio, 3.35% Alteromonas, 2.98% Flavobacteria, 2.57% Pseudoalteromonas, 1.96% Rhodobacteracea, with 57.59% being unprobed (Figure 3). In situ Montastraea cavernosa was 21.68% Endozoicomonas, 3.13% Vibrio, 1.98% Alteromonas, 2.29% Flavobacteria, 3.63% Pseudoalteromonas, 2.29% Rhodobacteracea, with 65% being unprobed (Figure 3). Mesocosm Diploria labyrinthiformis was 26.38% Vibrio, 16.29% Flavobacteria, 7.6% Endozoicomonas, 6.34% Pseudoalteromonas, 2.31% Alteromonas, 1.29% Rhodobacteracea, with 39.79% being unprobed (Figure 3). In situ Diploria labyrinthiformis was 29.98% Flavobacteria, 21.41% Vibrio, 10.11% Alteromonas, 5.68% Pseudoalteromonas, 5.5% Endozoicomonas, 1.15% Rhodobacteracea, with 29.16% being unprobed (Figure 3). There is no significant difference in bacterial composition across environments and species (R2= 0.04689, PERMANOVA, Figure 3). The data showed no significant difference in zooxanthellae between environmental variables (p= 0.8055, Kruskal Wallis, Figure 2).

Figure 1: Comparison of the microbial abundances of in situ (n=12) and ex situ (n=12) coral specimens of Diploria labyrinthiformis, Porites astreoides, and Montastraea cavernosa.

Figure 2: Comparison of the zooxanthellae abundances of in situ (n=12) and ex situ (n=11) coral specimens of Diploria labyrinthiformis, Porites astreoides, and Montastraea cavernosa.

Figure 3: Comparison of the bacterial composition of in situ (n=3) and ex situ (n=3) coral specimens of Diploria labyrinthiformis, Porites astreoides, and Montastraea cavernosa.
Discussion
Analysis shows that there was a significant difference between in situ and mesocosm microbial abundance across environments among all three coral species (Porites astreoides, Montastraea cavernosa, Diploria labyrinthiformis). This result confirms the expected outcome of bacterial abundances in this study. The environmental conditions within the mesocosm are sparsely different from those within in situ environments. Within the mesocosm, all environmental conditions are unchanging, which varies from ocean environments. For example, the temperature within the mesocosm is regulated and remains one exact degree all year-round, while in the field there is seasonality, which allows the temperature to change slightly. The light is also less variable within the mesocosm environment compared to in situ environments where light varies daily. Because the water in the mesocosm is regulated, this could limit the amount of nutrients that is entering the mesocosm system, forcing the corals to live in a low nutrient environment. The nutrient levels within the water column of the mesocosm and in situ environment could also be vastly different. This difference in nutrient levels could have a huge effect on nutrient consumption of the coral, similarly to how plant microbiota shifts in different soil types (Bulgarelli et al. 2013).
Prior research that has been conducted on the bacterial communities within the BIOS mesocosm and revealed that there was a lower abundance of bacteria within mesocosm compared to in situ environments (Han 2022). This could greatly affect the number of bacteria that the corals are uptalking when within the mesocosm, explaining why there is a much lower abundance of bacteria when compared to in situ specimens of the same species. The mesocosm corals are also not being feed zooplankton regularly while the in situ specimens have a wide abundance of zooplankton to feed on within the water column. It has been recorded that within the BIOS mesocosm, there is a much lower abundance of zooplankton compared to Bailey’s Bay (Han 2022). This could possibly influence how the bacterial comminutes are being regulated within the coral animal, lowering the bacterial abundance when within mesocosm environments. Because of this lack of zooplankton, the corals within the mesocosm could being heterotrophically feeding on the bacteria within their microbiome in efforts to replace the energy they would have been gaining from the zooplankton. This would lessen the bacterial abundance within the mesocosm corals, which has been observed.
Even though the bacterial abundance was drastically lower among mesocosm specimens compared to in situ corals, the composition and proportion of bacterial groups remained the same between mesocosm and in situ colonies. It has been suggested that corals can rapidly adapt to new environmental conditions by modifying the population size of their symbiotic bacteria (Reshef et al. 2006). This shows that in these coral species under this specific environment, the composition and proportion of different bacterial groups remained the same when within mesocosm environments. The results of this study also determined that there was no difference in bacterial composition proportions across species either. This was likely due to the small sample size and high standard deviation. These results contradict the expected outcome of bacterial composition in this study.
There was also a huge proportion of bacteria within each species that remained unprobed, meaning there could be differences within that proportion that can’t yet be determined. Wegley (2007) probed coral species Porties astreiodes to see the composition of the bacterial communities. They found that the most prominent bacteria phylum in the metagenome were Proteobacteria (68%), Firmicutes (10%), Cyanobacteria (7%) and Actinobacteria (6%) (Wegley et al. 2007). However, the present study probed for bacterial groups Pseudoalteromonas, Endozoicomonas, Rhodobacteracea, Alteromonas, Vibrio, which are all members of the phyla Proteobacteria (excluding Flavobacteria which is a part of Bacteroidetes), which made up 68% of the P. asteroids in Wegley’s study. This could explain the huge proportions of bacteria that were found as unprobed in our data. The remaining three phylum could be present in the unprobed for bacterial group. More probes would need to be conducted to see if these other groups would have differences between species and/environments.
Zooxanthellae was shown to be statistically insignificant between in situ and mesocosm environments, which suggests that zooxanthellae abundance does not change when within an aquaria environment. This contradicts the expected outcome of zooxanthellae density in this study. Photoadaptation was expected to be observed among the corals within the mesocosm setting because of the different light quality, but this was not seen. The mesocosm corals are most likely obtaining the correct light intensity to maintain a normal density of zooxanthellae within their tissue. Zooxanthellae can increase the corals susceptibility to oxidative stress which results from the production and accumulation of reactive oxygen species (ROS) and can severely damage lipids, proteins, and DNA (Franklin 2004; Roth 2014). This process is caused by the influence of to much light, which causes photoinhibition, which is when the rate of damage exceeds the rate of repair (Niyogi 1999). The corals within the mesocosm are most likely not experiencing this phenomenon because the density of zooxanthellae within their tissue remains the same as corals of the same species in situ.
This study was heavily limited by the four-week time frame. With more time, this study could have sampled more coral fragments for a larger sample size which would result in a better representation of each coral species. When preforming fluorescence in situ hybridization (FISH), there was only time to preform it on one coral from each species and environment (n=6), which highly limits the accuracy of the statistical tests being conducted on this data because of a higher standard deviation.
If this study had more time and resources, it would have benefited from the use of a larger sample size of each coral species within each environment (n=10). FISH analysis would also be performed in much more detail with a higher sample size for each species (n=10). There would also be more probing conducted of other bacterial groups along with Proteobacteria (Firmicutes, Cyanobacteria, Actinobacteria).
Conclusion
This study provides a small snapshot into the complex bacterial abundance and composition held within the coral microbiome. Studies like this are important because the microbial composition and abundance of corals could have a huge effect on their health. Coral health studies are typically preformed within ex situ environments and this study shows that there are vast differences between corals in situ and corals ex situ. This suggests that more research like this should be conducted to determine if these bacterial differences have an effect on normal coral biological functions to determine if corals need to be kept in aquaria environments differently than they are currently.
Acknowledgments
Alexandra Sinno would like to acknowledge Dr. Rachel Parsons for being there every step of the way during this study. A huge thank you goes to Dr. Samantha de Putron for mentoring Alex for the duration of this project and giving advice on the writing of this manuscript, proposal and final presentation. Christopher Spagnolia helped greatly with the use of RStudio and Alex thanks him for that. Lastly, Alex would like to give thanks to the Bermuda Institute of Ocean Sciences for the resources and facilities that made this research possible and the University of Rhode Island for allowing her to participate in this program.
References
Bourne, DG., Morrow, K. M., & Webster, N. S. (2016). Insights into the coral microbiome: underpinning the health and resilience of reef ecosystems. Annu Rev Microbiol, 70(1), 317-40.
Broderick, E., Bushaw-Newton, K., Santangelo, J., & Kim, K. (2008). Role of the coral surface microbiota in disease: an in situ test using the Gorgonia-Aspergillus pathosystem. In 11th International Coral Reef Symposium.
Bulgarelli, D., Schlaeppi, K., Spaepen, S., Van Themaat, E. V. L., & Schulze-Lefert, P. (2013). Structure and functions of the bacterial microbiota of plants. Annual review of plant biology, 64, 807-838.
Chu, ND., & Vollmer, S. V. (2016). Caribbean corals house shared and host‐specific microbial symbionts over time and space. Environmental microbiology reports, 8(4), 493-500.
Chornesky, EA., & Peters, E. C. (1987). Sexual reproduction and colony growth in the scleractinian coral Porites astreoides. The biological bulletin, 172(2), 161-177.
Falkowski PG, Dubinsky Z, MuscatineL, McCloskey LR. (1993). Population control in symbiotic corals. Bioscience 43:453–64
Franklin, D. J., Hoegh-Guldberg, P., Jones, R. J., and Berges, J. A. (2004). Cell death and degeneration in the symbiotic dinoflagellates of the coral Stylophora pistillata during bleaching. Mar. Ecol. Prog. Ser. 272, 117–130.
Galand, PE., Chapron, L., Meistertzheim, A. L., Peru, E., & Lartaud, F. (2018). The effect of captivity on the dynamics of active bacterial communities differs between two deep-sea coral species. Frontiers in Microbiology, 9, 2565.
Garren M, Azam F. (2012). Corals shed bacteria as a potential mechanism of resilience to organic matter enrichment. ISME J. 6:1159–65
Kimes NE., Van Nostrand, JD., Weil, E., Zhou, J., Morris, PJ. 2010. Microbial functional structure of Montastraea faveolata, an important Caribbean reef-building coral, differs between healthy and yellow- band diseased colonies. Environ. Microbiol. 12:541–56
Kooperman N., Ben-Dov, E., Kramarsky-Winter, E., Barak, Z., and Kushmaro, A. (2007). Coral mucus-associated bacterial communities from natural and aquarium environments. FEMS Microbiol. Lett. 276, 106–113.
Lema KA, Willis BL, Bourne DG. (2012). Corals form characteristic associations with symbiotic nitrogen- fixing bacteria. Appl. Environ. Microbiol. 78:3136–44
Niyogi, K. K. (1999). Photoprotection revisited: genetic and molecular approaches. Annu. Rev. Plant. Physiol. Plant. Mol. Biol. 50, 333–359.
Palardy JE., Rodrigues, LJ., Grottoli, AG. (2008). The importance of zooplankton to the daily metabolic carbon requirements of healthy and bleached corals at two depths. J. Exp. Mar. Biol. Ecol. 367:180–88
Porter K. G., & Feig, Y. S. (1980). The use of DAPI for identifying and counting aquatic microflora 1. Limnology and oceanography, 25(5), 943-948.
Pratte ZA., Richardson, L. L., and Mills, D. K. (2015). Microbiota shifts in the surface mucopolysaccharide layer of corals transferred from natural to aquaria settings. J. Invertebr. Pathol. 125, 42–44.
Ravindran J., Kannapiran, E., Manikandan, B., Francis, K., Arora, S., et al. (2013). UV-absorbing bacteria in coral mucus and their response to simulated temperature elevations. Coral Reefs 32:1043–50
Reshef, L., Koren, O., Loya, Y., Zilber‐Rosenberg, I., & Rosenberg, E. (2006). The coral probiotic hypothesis. Environmental microbiology, 8(12), 2068-2073.
Ritchie KB. 2006. Regulation of microbial populations by coral surface mucus and mucus-associated bacteria. Mar. Ecol. Prog. Ser. 322:1–14
Rohwer F., Seguritan, V., Azam, F., & Knowlton, N. (2002). Diversity and distribution of coral-associated bacteria. Marine Ecology Progress Series, 243, 1-10.
Roth, M. S. (2014). The engine of the reef: photobiology of the coral–algal symbiosis. Frontiers in microbiology, 5, 422.
Röthig T., Roik, A., Yum, L. K., and Voolstra, C. R. (2017). Distinct bacterial microbiomes associate with the deep-sea coral Eguchipsammia fistula from the Red Sea and from Aquaria Settings. Front. Mar. Sci. 4:259.
Schöttner S., Hoffmann, F., Wild, C., Rapp, H. T., Boetius, A., and Ramette, A. (2009). Inter-and intra-habitat bacterial diversity associated with cold-water corals. ISME J. 3, 756–759.
Siboni N, Ben-Dov E, Sivan A, Kushmaro A. (2008). Global distribution and diversity of coral-associated Archaea and their possible role in the coral holobiont nitrogen cycle. Environ. Microbiol. 10:2979–90
Stefels J. (2000). Physiological aspects of the production and conversion of DMSP in marine algae and higher plants. J. Sea Res. 43:183–97
Van Alstyne KL, SchuppP, Slattery M. (2006). The distribution of dimethylsulfoniopropionate in tropical pacific coral reef invertebrates. Coral Reefs 25:321–27
Wegley L., Edwards, R., Rodriguez‐Brito, B., Liu, H., & Rohwer, F. (2007). Metagenomic analysis of the microbial community associated with the coral Porites astreoides. Environmental microbiology, 9(11), 2707-2719.
Wild C, Huettel M, Klueter A, Kremb SG, Rasheed MYM, Jorgensen BB. (2004). Coral mucus functions as an energy carrier and particle trap in the reef ecosystem. Nature 428:66–70
Yellowlees D, Rees TAV, Leggat W. (2008). Metabolic interactions between algal symbionts and invertebrate hosts. Plant Cell Environ. 31:679–94